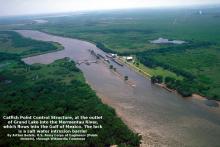
Saltwater intrusion is the flow of seawater into fresh water water bodies like rivers or aquifers due to natural processes or human activities. It is a major concern for most coastal areas as it can induce contamination of water resources and therefore drinking water, and may cause damage to agriculture. Saltwater intrusion, through surface or ground water sources, may diminish the availability and/or quality of source waters for drinking water utilities and irrigation. It is also a concern for the quality of coastal ecosystems as it may undermine the conditions of vegetation and therefore the stability of dunes, and may compromise coast wetlands, therefore impacting their ecosystem services, with implications in coastal socio-economy. An explanation of the dynamics of saltwater intrusion is provided in this video.
Saltwater intrusion is driven by both natural and anthropogenic pressures. Natural drivers include storm surges, hurricanes, climatic variability, sea level rise, and subsidence (see Figure 1). Anthropogenic drivers include anthropogenic climate change which may cause sea level rise and changes in wave forcing, ground water pumping, reduction in river discharge from dam construction, river barrages or water withdrawal or diversion, land drainage, and land-use changes within catchments (see Figure 2). Spatial and temporal scales of drivers are markedly different. Chronic drivers act over very long time scale (up to tens of centuries) while acute drivers operate on very short time scales, down to minutes in the case of extreme meteorological events. Tsunamis are known to be important stressors for saltwater intrusion. For instance, the tsunami that occurred in Southern Asia on December 26th 2004, caused widespread destruction and contamination of coastal aquifers. Seawater filled domestic open dug wells and also entered the aquifers via direct infiltration during the first flooding waves and later as ponded seawater infiltrated through the permeable sands that are typical of coastal aquifers. In Sri Lanka alone, it is estimated that over 40,000 drinking water wells were either destroyed or contaminated (Illangasekare et al., 2006).
Figure 1. Schematic representation of the spatial and temporal scales of natural saltwater intrusion drivers. Temporal scale varies from fast-acting and low frequency (i.e., acute) to slow-acting and consistent (i.e., chronic). Spatial scale indicates the size of the impacted area. From White and Kaplan (2017).
Figure 2. Schematic representation of the spatial and temporal scales of anthropogenic saltwater intrusion drivers. Many anthropogenic drivers act over a wide variety of spatiotemporal scales as a function of development intensity (e.g., local agricultural water abstraction in a small river vs. damming a large river). From White and Kaplan (2017).
Drivers of saltwater intrusion are schematically presented in Figure 3, where one can note the change in the coastal environment that is managed by humans, who tend to make it more regular, the lower level of groundwater resources due to pumping and the corresponding expansion of the saltwater body towards the inlands.
Figure 3. Ecosystem change after saltwater intrusion. Modified after Sweetian (Own work) [CC BY-SA 3.0 (https://creativecommons.org/licenses/by-sa/3.0)], via Wikimedia Commons.
At the coastal margin, fresh groundwater flowing from inland areas meets with saline groundwater from the ocean. A schematic representation of the saltwater-freshwater interaction and water flows in the inlands is given in Figure 4. Freshwater flows from inland areas in the form of surface runoff (river flow and overland flow from impervious areas) and groundwater bodies if these are present. A necessary condition for the presence of groundwater bodies is marked permeability of coastal soils, which receive water from infiltration, and store it in a dynamic reservoir which releases water into the sea through a slowly moving water flow. We assume in Figure 4 that such permeable soil exists. When the coastline is formed by impervious rocks the situation is simpler and saltwater-freshwater interaction takes place along rivers only. Because rocky coasts are often steep, and therefore river beds are markedly sloped, saltwater intrusion is generally limited in these cases.
Figure 4. Saltwater-freshwater interaction
Groundwater flow takes place at the microscopic scale through movement of water particles along soil pores. As such, it is typically a 3-dimensional (3-D) flow, which is impossible to describe mathematically through a deterministic model. Stochastic models are thus typically used. If we focus on the flow properties averaged over sufficiently spatial scale, the groundwater flow can generally be efficiently represented by using 1-dimensional (1-D) models, under the assumption that the velocity vector for each water particle does not change along two spatial directions. This assumption implies that the water flow is gradually varied, that is, that the stream lines are sensibly rectilinear and parallel. It follows that the distribution of pressure in each cross section follows the hydrostatic law.
Under the above assumptions, groundwater flow takes place according to Darcy's law, which states that the volumetric flow rate for a 1-D water flow is a function of the flow area, potential energy, fluid pressure and a proportionality constant. The law was formulated by Henry Darcy based on the results of experiments on the flow of water through beds of sand. According to Figure 5, Darcy's law states that the total discharge, Q (units of volume per unit time) is equal to the product of the hydraulic conductivity of the medium, κ (units of velocity), the cross-sectional area to flow, A (units of area), and the gradient along space of the hydraulic head J (adimensional), namely:
Q = k A J = k A ∂H/∂x
where x is the flow curvilinear coordinate (note that flow is 1-D and therefore H varies along x only) and H is hydraulic head.
Water flows in the direction of decreasing head and therefore the head itself determines whether the flow is directed towards inland or the sea. The hydraulic head represents the energy of flow for unit weight of water. Under the assumption of very limited velocity of the flow, the hydraulic head is the sum of elevation head and pressure head. Namely, for a given water flow a groundwater body, the hydraulic head of an arbitrary fluid particle in a given flow cross section is given by
H = z + p/γ
where z is the elevation of the fluid particle itself with respect to a reference level and γ is the specific weight of the fluid. The above mentioned hydrostatic law suggests that H is constant in each cross section of the water flow itself. Therefore, with reference to Figure 5, the water flow can be computed as
Q = k A (h3 -h4)/L
Figure 5. Schematic illustration of Darcy's experiment. Modified after https://commons.wikimedia.org/w/index.php?curid=1294394
In coastal aquifers we can generally observe a flow of freshwater that comes from inland, which is fed by infiltration from rainfall or rivers (see Figure 4), and an infiltration of saltwater from the sea, which is pushed towards inland by its hydraulic head and, in some cases, by energy associated to wave motion or other forcings. Therefore an interface is established in the soil. If the hydraulic head of the aquifer is lower than that of the seawater, the latter will intrude in the land, otherwise a freshwater flow into the sea will occur (see Figure 6).
Figure 6. Saltwater-freshwater flow. By Lamiot (Own work) [CC BY-SA 3.0 (https://creativecommons.org/licenses/by-sa/3.0)], via Wikimedia Commons
We assume that aquifer and sea are in steady state conditions and there is no mixing at the interface between salt water and fresh water. A simple application of hydrostatics leads to determining the position of the interface between salt water and freshwater. Saltwater has a higher content of dissolved salts and minerals and thus it is denser than freshwater therefore inducing a higher hydraulic head. An analytical relationship was independently proposed by Badon Ghijben and Herzberg, by taking into account that salt and freshwater have different densities. We refer to Figure 7 and impose that a fluid particle in laying equlibrium on the surface separating salt water-fresh water is subjected by equal pressure from salt water, with head H, and fresh water with head H+h. Therefore, one obtains:
γsH = γf(H+h)
therefore obtaining
γsH = γfH+γfh
that is,
H = (γf/(γs - γf)) h
namely
H = α h .
α is given by ρf/(ρs - ρf) where ρs and ρf are the densities of salt and fresh water, respectively. If we assume that ρs=1025 kg/m3 and ρf=1000 kg/m3 then one obtains
H = 40 h .
Given that the height of the water table generally increases while moving inland, saltwater moves into coastal aquifers in a wedge shape under the freshwater. Ordinarily the inland extent of the saltwater wedge is limited because fresh groundwater levels, or the height of the freshwater column, increases as land elevation gets higher.
Figure 7. The Badon Ghijben-Herzberg principle: a fresh-salt interface in an unconfined coastal aquifer.
The interface models given by the Badon Ghijben-Herzberg principle provides a coarse approximation of what actually happens in a coastal aquifer systems. In fact, the underlying assumptions are not met in practice and therefore some restrictions on the applicability of the principle should be considered:
- First, the brackish zone between fresh and saline groundwater should only be schematised by an interface when the thickness of the brackish zone is very limited. This condition applies only in rare situations where the freshwater lens receives significant recharge.
- Second, the principle assumes a hydrostatic equilibrium, whereas in reality the aquifer system might considerably deviate from this equilibrium situation. In those cases, e.g. in freshwater bodies near the shoreline, the Badon Ghijben-Herzberg principle should not be applied, because the computed position of the interface deviates from the actual position as the coast is approached.
Actually, in many coastal aquifer systems, a relatively broad transition zone (or mixing zone, zone of dispersion or brackish zone between fresh, brackish and saline groundwater) is present because of various processes during geological history (Oude Essink, 2003; see Figure 8).
Figure 8. Freshwater and saltwater mix in the zone of dispersion by the processes of diffusion and mechanical dispersion (Figure modified from Cooper, H. H., 1964, A hypothesis concerning the dynamic balance of fresh water and salt water in a coastal aquifer: U.S. Geological Survey Water-Supply Paper 1613-C, p. 1-12).
Therefore, to make the interpretation more realistic more sophisticated models are required that take into account variable densities and the transport of solutes. These models are referred to as solute transport models or salt water intrusion models. They apply the advection-dispersion equation and can simulate, among others, salt water intrusion in coastal aquifers where mostly non-uniform density distributions occur. Also, 2-D or 3-D models may be used.
Factors that affect the saltwater - fresh water interface may be natural or human induced. They may be classified according to their spatial scale, ranging from global to regional and local.
Global scale factors include:
- Rising of sea water level; it may be caused by land subsidence (relative sea level raise), ice cap melting after climate change and thermal expansion of sea water after global warming.
- Change in precipitation: a slower infiltration rate into aquifer to replenish the amount of fresh water may induce a lowering of the water table.
- Increased evapotranspiration: it may be due to global warming (but there is no consensus on the the effect of global warming on evapotransiration) or increased irrigation (in this case it is a local or regional factor).
Regional scale factors include:
- Subsidence: it may be due to oil and gas extraction, water pumping, or soil compaction.
- Local climate change: it may be due to heat island effects or other local forcings.
- Urbanisation: it reduces the recharge of aquifers.
Local scale factors include:
- Change in soil permeability: due to the construction of barriers or soil compaction.
- Increased Consumption of Water: increased water demands for civil and agricultural use.
- Water pumping: it is a very frequent determinant of saltwater intrusion. Pumping lowers the level of the water table. Saltwater can contaminate freshwater resources by interfering with the wells therefore increasing the salinity of drinking water (see Figure 9).
Figure 9. Effect of pumping on freshwater and saltwater interaction. Credit: The National Environmental Education and Training Foundation (NEEF).
The effect of pumping is particularly concerning as many aquifers in the world are overexploited. A comprehensive assessment of groundwater exploitation in the United States is given here. A schematic diagram of the various pathways for saltwater intrusion in the aquifers of southwest Florida is shown in Figure 10.
Figure 10. Schematic diagram of the various pathways for saltwater intrusion in the aquifers of southwest Florida. They include (1) encroachment from the ocean, (2) infiltration from tidal marshes, estuaries, and bays, (3) leakage from unprotected canals, (4) upward leakage from deeper aquifers, and (5) movement of residual saltwater from previous sea-level high stands. From Prinos (2013).
A nice summary of how groundwater exploitation may induce intrusion of saline water is given in this video.
Monitoring is essential to prevent and mitigate the impact of saltwater intrusion. It can be carried out by using direct and indirect methods. Direct methods include measurement of groundwater salinity profiles and groundwater sampling of observation and active wells. Direct methods were mostly used to detect groundwater salinity up to recent times. Indirect methods are mostly based upon geophysical techniques such as ground penetrating radar. The device produces a continuous cross-sectional picture of shallow subsurface conditions. Measurement of electrical conductivity is also widely used. Observations indicate the concentration of dissolved solids that are present as ions (charged molecules) in a polar solution like water. In a mix of seawater with freshwater for example, chloride is typically one of the predominant ions present, therefore electrical conductivity can be used as a relative measure of chloride concentration.
Saltwater monitoring programs include the definition of monitoring methods and objectives, design of field surveys to characterize possible seawater intrusion, sample collection and data analysis. Monitoring of contaminated aquifers is an integral part of the activity related to the modern human–environment interaction. Its goal is to detect and characterize changes in the aquifer system that might arise from human influence and natural causes on aquifer systems. Seawater intrusion is a typical result of such a human interaction (Melloul and Goldenberg, 1997).
As an example, Figure 11 shows the location of salinity monitoring station along the delta of the Po River in 2012, while Figure 12 shows the corresponding map of salinity resulting from the measurements.
Figure 11. Location of salinity monitoring station along the delta of the Po River in 2012.
Figure 12. Water salinity along the delta of the Po River in 2012. PSU means Practical Salinity Units, which is the ratio between electrical conductivity of the sampled water and that of a standard freshwater sample (which by definition contains 32,4 grams of salt dissolved in 1 kg of solution at the temperature of 15 °C).
Restoration after salt water intrusion is difficult and expensive and needs to be based on the preliminary identification causes, damage, ecological and societal value of the affected areas. In particular, the following questions need to be addressed:
- What are the drivers of salt water intrusion? Are they anthropogenic, natural or both?
- Which ecosystem structures and functions are degraded and to what extent?
- To what ecological condition the ecosystem should be restored?
- Can the drivers of degradation be modified? What means are available to get to target?
The subsequent step is the definition of the restoration goals. Setting goals is critical for determining project successes and learning from failures, but can be problematic for coastal ecosystems impacted by natural and anthropogenic salt water intrusion. Particularly challenging is the assessment of the vulnerability and/or resilience of restored sites to present and future environments.
Efforts to recover from salt water intrusion fall into three overarching categories:
- modification of groundwater and surface water withdrawals;
- modification of groundwater and surface water deliveries;
- using engineered structures.
An example of engineered structure is the cut-off, which may consist of an an excavated trench backfilled with material of limited permeability like clay. They may also consist of steel sheet piling, vinyl sheet piling or bentonite mats.
Table 1 present a synthesis of strategies, approaches, and tools to mitigate the impacts of saltwater intrusion in coastal regions (from White and Kaplan, 2017).
Table 1. Strategies, approaches, and tools to mitigate the impacts of saltwater intrusion in coastal regions; references are given listed in the gable can be found in White and Kaplan (2017)).
All approaches rely to some extent on the use of models to develop alternative management scenarios. Interventions often introduce strong controls and regulation of the drivers. One drawback of this approach is that the natural variability of the ecological regime of the affected systems is reduced, therefore reducing the resilience of the system as well. This is an implication that needs to be carefully explored.
Ultimately, a choice need to be made between restore or retreat, or a combination of the two. In some cases a well designed retreat may be preferable, allowing the restoration of the original habitat therefore providing the means to avoid an excessive control on the ecology, thereby promoting the restoration of a diverse environment. The optimal choice depends on local condition and the socio-ecologic value of the interested areas.
White E., and D. Kaplan. 2017. Restore or retreat? Saltwater intrusion and water management in coastal wetlands. Ecosystem Health and Sustainability 3(1):e01258. doi: 10.1002/ehs2.1258
Illangasekare, T., et al. (2006), Impacts of the 2004 tsunami on groundwater resources in Sri Lanka, Water Resour. Res., 42, W05201, doi:10.1029/2006WR004876.
Essink, G. H. O. Mathematical models and their application to salt water intrusion problems. TIAC, 3, 57-77.
Melloul, A. J., and Goldenberg, L. C. (1997). Monitoring of seawater intrusion in coastal aquifers: basics and local concerns. Journal of Environmental Management, 51(1), 73-86.
The Powerpoint presentation of this lecture
Last modified on April 18, 2017
- 400 viste